Marc Grey
Despite being one of the safest types of energy generation, there is a common perception that nuclear power is dangerous. Probably the most common view about nuclear power danger is that a reactor may explode. In this article, we explore what type of explosions can occur and along the way cover some misconceptions and confusions on this topic. We will also briefly look at how some of these explosive causal factors might be mitigated.
Before looking at explosions, it is useful to understand the basic components of a typical commercial nuclear reactor. This was covered in a previous article but will be briefly repeated here. The most common commercial reactor is a ‘Light Water Reactor’ (LWR), which can be thought of as ‘nuclear steam engine’:
- a core is composed of fuel (usually uranium 235 and 238 oxides) encased in rods (neutron transparent zirconium)
- fuel in the core generates heat by a controlled fission reaction (neutron impact, nucleus splitting and neutron release)
- water flows through the core in pipes which removes heat and moderates (slows) neutrons to increase impact probability
- the core and coolant are enclosed in a pressure vessel
- control rods made of a neutron absorbing material can be positioned close to the fuel rods to control the fission reaction
- the entire system is enclosed in a containment structure (usually concrete)
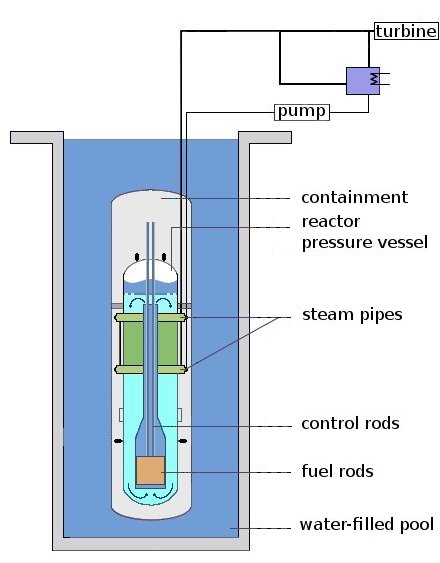
The first and most obvious source of an explosion is steam over-pressurisation. This is not unique to nuclear reactors; any pressurised steam system can burst its pressure vessel explosively if too much pressure builds up. In this case there are two basic reasons why this can happen 1) too much heat being generated by the core or 2) insufficient heat removal by the water coolant. We will cover 1) below, but 2) is usually caused by coolant leaks or flow failure (e.g. power loss to pumps).
The next possible source of an explosion is the possibility that water can be split into hydrogen and oxygen. This is made possible because of zirconium which, if heated enough, acts as a catalyst. The oxygen is not such a problem but hydrogen surely is, and a hydrogen explosion is another way to burst the pressure vessel containing the core.
The last source is the behaviour of the core itself. It is possible to lose control of the fission reaction, and suffer what is usually called a ‘criticality excursion‘.
To understand what this means, a brief digression covering ‘neutron economy’ is needed. Neutrons are produced by fissioning nuclei, and can either go on to fission new ones, or escape the core or get absorbed by control rods etc. The number of neutrons created vs the number lost or absorbed by control rods etc is called the reactivity and is usually expressed as a number range:
- zero means all the neutrons produced are used in fission events, so the fission reaction is stable. This is called ‘critical’
- positive means there are excess neutrons produced and the number of fission events will tend to increase exponentially. This is called ‘super critical’
- negative means there are insufficient neutrons produced and the number of fission events will tend to decrease. This is called ‘sub critical’
Generally a nuclear reactor core is kept at a ‘critical’ state by moving the control rods. Transient ‘super’ or ‘sub’ criticality can occur when altering the reactor power level is desired. E.g. withdrawing the control rods a little will make the reactor go briefly super critical before settling down to critical at a higher power level.
Completely removing all (or sometimes just a few) control rods may make a reactor go uncontrollably supercritical. This is called a ‘criticality excursion‘: essentially the core is undergoing what happens in a nuclear weapon initialisation. However, the core cannot explode with the same violence as a weapon because it does not have the implosive containment needed to keep the core from melting or blowing itself apart before all its fuel is fissioned (a reactor cannot explode like a nuclear weapon)!
We will consider some real life examples to illustrate the various scenarios:
The small 1 MW research reactor SL-1 was sent supercritical on 3 January 1961 when an operator completely removed the central control rod. Almost immediately the heat generated by the excursion explosively vaporised the core and the resulting pressure wave caused a water hammer that made the pressure vessel jump approximately 3 metres upward. While the vessel was not destroyed, pipes, connections and control rod attachment points were, and high pressure steam and water was sprayed into the containing room along with radioactive debris from the wrecked core.
In summary we have essentially a pure core supercritical event with collateral damage.
The huge Chernobyl 1 GW number 4 reactor was sent supercritical by a combination of operator actions, management overrides and reactor design flaws on 26 April 1986. This reactor was not a LWR but a Soviet designed RBMK which had the fuel encased in ‘channels’ that were water cooled but graphite moderated, with no containment building. While the design had some nice features (it could be refueled while running) it had some serious flaws (instability at low power). Unfortunately during a test procedure the reactor was allowed to run at very low power with most control rods withdrawn. Due to the low power instability, some parts of the core went super critical and could not be controlled even with an emergency shutdown. The heat released vaporised the cooling water and ruptured many channels in a massive steam explosion. The lack of a reinforced containment building meant that radioactive debris were ejected into the surrounding air.
In summary, the core excursion triggered a steam explosion, which, due to lack of a proper containment building, released a large amount of radioactive debris. This is regarded as the worst nuclear accident to have ever occurred.
The Fukushima Daiichi 460-780 MW number 1-3 reactors suffered core meltdowns between 12 and 15 March 2011. The Tohoku quake and tsunami took out grid and backup power sources, which resulted in cooling pump shutdown. Despite shutting down the reactors, the buildup of decay heat caused core overheating. The massive heat buildup melted the fuel rods and other core components. In all 3 reactors the core became hot enough to melt through the pressure vessel and fall onto the floor of the enclosing containment building. The catalysing effect of hot zirconium split hot steam into hydrogen and oxygen which vented from the pressure vessel into the containment area, where 3 hydrogen-air explosions occurred. Containment for reactors 1 and 3 were breached, releasing radiation into the outside air.
In summary these were core meltdowns that breached their pressure vessels by melting through them rather than exploding, and then produced hydrogen which exploded inside the main containment structures – damaging them. This is regarded as the second worst nuclear accident.
Now that we’ve shown the types of explosive events that can happen to reactors (and illustrated one that cannot), we will examine them from a safety centric point of view.
The first two examples point clearly to the need for safety culture as discussed in an excellent article published previously. In the case of SL-1 it was documented to not withdraw the centre control rod! In the case of Chernobyl there were numerous instances of safety protocols switched off during the test run. In addition, the instructions for doing the test required the reactor to be at least 700 MW output – and this was ignored.
Keeping with these first two again, reactor design and configuration is a big factor. SL-1 used 93% enriched uranium 235 as a fuel so it went super critical immediately when the centre control rod was removed. This is clearly not a passive safe design! In the case of Chernobyl, the low power instability was a terribly dangerous design choice which meant that even though it ran on non-enriched uranium fuel it could still go super critical under some circumstances! Couple that with the lack of a secondary containment structure and, well what were they thinking?
Next, looking at Fukushima, we see that even a much safer design (LWR, low enrichment fuel, stable power characteristic, secondary containment) can be nearly as bad as an obviously dangerous one. So the core did not go super critical – but melting through the pressure vessel and creating a hydrogen explosion inside the secondary containment was very serious. In addition, TEPCO (plant operator) were aware of safety issues around tsunamis and had not implemented changes. Also, there were contemporary reactor designs that could power themselves if the grid failed, which would have avoided this disaster. This shows the danger of complacency and lack of desire to constantly improve.
A big takeaway from all this is that using water for cooling or moderation is not a great idea! In particular with the use of solid fuel encased in zirconium this is an explosion waiting to happen. In a previous article, the point was made that in the 1950s the inventor of the LWR said pretty much the same thing! He proposed using liquid fuel – thorium dissolved in molten salt – to avoid the need for water, zirconium or pressure.
One final note to take away from the SL-1 case: a lower power reactor (say 10-100 MW) can get into the worst possible situation, but the pressure vessel (and secondary containment if added) may be able to handle the forces involved and contain the situation. Whereas scale that to 1 GW and there is no chance! This idea is being actively researched under the title ‘Small Modular Reactors’ or SMR.
So in conclusion, it does look like there are several ways to mitigate the risk of any type of explosive event. This is good news, as nuclear is really the only viable option if we are globally being coerced into abandoning fossil fuels.
If you enjoyed this article please share it so others can discover The BFD.